Next: About this document ...
Chapter 31
Nuclei and Radioactivity
As a consequence of Rutherford's experiment it was realized that most of the mass of atoms is localized in a very small
volume, i.e. the atomic nucleus. Moreover it was clear that the nucleus contained an amount of positive charge
equal to the negative charge carried by the electrons, and soon the actual carrier of the positive charge was identified
and named proton (greek root: protos = the first, think e.g. of prototype, protagonist, etc.). This realization
suggested that there must be a completely new force at play within the nucleus, since otherwise the strong
electrostatic repulsion between positive charges would prevent the nucleus from holding together. This force was
named the nuclear force. Today we know there are two types of nuclear forces, the strong
nuclear force, which is responsible for holding the nuclei together (and it is in fact about 100 times stronger than the
electric force) and the weak nuclear force, which intervenes in many radioactive decays (more on the weak force in
the next lectures).
But another early realization was that, to account for the mass of the nucleus, one needed to have much more mass than
what was given by a number of protons equal to the number of electrons. The logical hypothesis in those early days was to
assume that, within the nucleus, the actual number of protons was larger than the corresponding number of orbiting electrons,
but the nucleus also contained a certain number of electrons, in the right quantity to balance the excess positive charges.
It was not until 1932 that the neutral counterpart of the proton, the neutron was discovered, showing
that the nucleus contains a number of protons equal to the number of orbiting electrons plus a certain amount of
neutrons to account for the extra mass.
Question : why did it take 20 years to discover the neutron ?
To summarize our understanding of atoms and nuclei :
- an element is identified by the number of protons in the nucleus. This is also equal (under normal conditions) to the
total number of orbital electrons. But be aware that the atom of an element can lose or acquire one or more electrons (i.e.
become ionized) and still remain the same element (a Sodium ion is still considered to be Sodium), but if an
element loses or acquires a proton it becomes a different element.
- given that the chemical properties of an element are determined by the number (and organization) of electrons, then it is
also correct to say that the chemical properties are determined by the number of protons.
- on the other side, the number of neutrons is not uniquely identified by the element type. An element can have
several isotopes (= same place, since they occupy the same place in the Periodic Table), i.e its nucleus can
contain a variable number of neutrons. Isotopes are chemically identical, therefore it is very hard to separate them,
the only handle being the mass difference.
- to fully identify a nucleus, one has to specify its number of protons (which effectively tells us what element we are
dealing with) and the number of neutrons (that will tell us which isotope of that given element we have). In practice, the
standard notation is to give the number of protons (=Z = Atomic Number) and the sum of protons + neutrons (=A = Atomic Mass),
e.g.
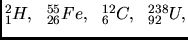
etc.
(notice that in principle it should not be necessary to specify Z, since this is already uniquely identified by the element's
symbol).
Nuclear Forces and Nuclear Stability. Let us form the simplest isotope, by joining a proton with a neutron. This is
(technically) still Hydrogen, even though one normally refers to it as Deuterium and even assigns to it its own
chemical symbol D. Deuterium is actually found in nature (in small quantities), usually in the form of heavy water which
is not
but
. Suppose you now take a nucleus of Deuterium (i.e 1 proton and 1 neutron bound together), measure
its mass and compare it with that of the original proton + neutron: what you will find is that the mass of the bound state
is less than that of the combined proton + neutron, when measured as free particles.
(Parenthesis : how do you measure the mass of sub-atomic particles?
answer: it depends on whether the particle is charged or neutral. If it is charged, its deflection in a magnetic field will
measure its momentum p=mv. One then needs an independent measurement, either of v or of the kinetic energy 1/2mv
, and m can
be extracted combining the two results. It is much harder for neutrals, typically the only way is to collide the neutral
particles with charged ones, and infer the neutral mass by means of energy-momentum conservation)
But, going back to our Deuteron,
what happens to the missing mass? One way to look at it is that the mass was transformed into (nuclear) energy, and this
energy is in fact the one the keeps the two particles bound together. Another (maybe easier to digest) way to look at it is
that when the two particles are staying together they do not have enough mass to produce the free particles.
Therefore, in order to split them, I would need to provide an amount of energy equal at least to the missing mass or mass
defect. Conversely, I can expect that, when I force the two particles together, some energy is liberated in the process,
specifically an amount of energy equivalent to the mass defect (we will come back onto this point, which is the key to the
understanding of nuclear power). Every single isotope does have a certain amount of missing mass (if it didn't, it could not
hold together as a nucleus, but it would be immediately torn apart by electrostatic repulsion between protons).
We could make a
plot of the amount of missing mass (more precisely the missing mass per proton or neutron, i.e. the total missing mass divided
by the number of protons + neutrons) versus atomic number and see that it has a maximum in correspondance to the nucleus of Iron.
From this we can derive two tentative conclusions:
- iron is the most stable nucleus
- there will be some difference in behaviour when we join or split nuclei that are lighter or heavier than iron (we will
come back onto this, which will be the key in understanding the difference between fusion and fission).
Nuclear Forces and Nuclear Instability.
Not all possible arrangements of neutrons and protons can be stable, and in fact it is a well known fact that several isotopes
of any element can only "live" for a finite time, after which they will undergo radioactive decay. In fact the heavier is the
nucleus the harder it is to achieve a stable configuration. All elements heavier then Bismuth (Z=83) do not have any stable isotope
at all.
Historically, the process of nuclear decay, when first observed in 1892, was thought of as the emission of some mysterious
radiation on the part of certain substances, and the phenomenon was called radioactivity. And, from the early days, it
was recognized that three types of radiation could be emitted, which, in lack of better understanding, were named
and
. In the early days it was known that
rays were positively charged (with twice the electron charge) they were massive and consequently not very
penetrating (could be stopped even by a thick piece of paper)
rays were negatively charged, light and very penetrating
rays were neutral and even harder to stop than
Today we have a much deeper understanding of the phenomena, specifically :
rays are really Helium nuclei, i.e. a bound state of two protons and two neutrons. When a nucleus is in
an unstable configuration, rather than just spitting out individual protons or neutrons, it likes to spit out a whole
particle. In doing so it will transmute itself into a different element, two steps down the Periodic Table (and also
with two less neutrons than the original nucleus)
rays are nothing but ordinary electrons. The actual process that takes place (within the nucleus) in
decay
is in fact a neutron decay :
(we will ignore the (anti)neutrino for the time being). This process too has the effect of transmuting the original element into
a different one, but this time, since we are effectively gaining an extra proton, we move one step up the periodic table.
Another type of
decay can also occur in some isotopes, when, instead of a negative electron, its antimatter
counterpart, the positron (= positive electron) is spat out. The reaction that actually occurs within the
nucleus is
NB: given that the mass of a free neutron is larger than the combined mass of (proton+electron),
decay of free neutrons can (and does) occur routinely. On the other side, because of energy-mass conservation, a free proton
canot decay into neutron+positron. But, within the nucleus, the proton can borrow (steal?) some energy from the nucleus (
therefore increasing the nucleus "mass defect") and give itself enough mass to be able to perform the transformation...
Positive
decay is exploited in PET (Positron Emission Tomography) scans: if a
emitter is introduced into the
body, emitted positrons will readily annihilate with regular electrons (this is what antimatter likes to do when it encounters
matter), producing pure energy, i.e. two back to back (because of momentum conservation) high energy photons.
Detection outside the body of the emitted photons allows to determine where the annihilations are taking place
(it is like a reversed X-ray, with the photon source being inside rather than outside the body...)
Question : what is the minimum energy these photons will have?
rays are just very high energy ( around 1 MeV = 1 million electron volts) photons.
emission does not
represent an actual nucleus decay, but just the rearrangement of a nucleus that was left in an unstable state because of
or
decay. Think of a card castle : it is a very unstable configuration and, if you remove a card, it will
collapse to the state of lowest gravitational potential energy.... But the difference in energy has to be accounted for: when a
nucleus drops down to a lower energy state, the difference in energy is emitted in the form of electromagnetic
radiationIt is a process analogous to the emission of photons by atomic electrons, but, in case of the nuclei,
the energies involved are much larger than for atomic electrons.
Life and families
Like all atomic and sub-atomic process, the time of occurrence of a nuclear decay cannot be predicted exactly, but only its
probability of occurring within a given time interval can be given. This probability is usually expressed in terms of the
half-life, i.e. the time after which, in average, half of an initial sample of a radioactive substance would have
decayed. Half-lives can be as short as a fraction of a second or as long as billions of years. Obviously a very unstable isotopic
configuration will have a very short lifetime, while the lifetime will be very long for a more stable configuration.
Rather than in terms of half-life, the decay of unstable particles is more generally described by physicists in terms of an
exponential function
with
= decay constant or
= lifetime. This expression allows to compute the remaining sample after any
arbitrary time t, not just for an integer number of half-lives. Half-life and lifetime are related through a fundamental property
of the exponential :
- linear function : equal differences for equal intervals
- exponential functions : equal ratios for equal intervals
. Consequently, any choice of
and
satisfying
will correspond to the same
interval
,
i.e, in our case, to the same time interval
.
One can immediately find the relation between half-life
and lifetime
: if at t = 0 one has
particles,
and this number is halfed at t = T
, one has :
Most of the knowledge we have about radioactive isotopes comes from experiments with accelerators, where different
nuclei are smashed together. But we also know that there are several radioisotopes found in the environment, and some of them
have relatively small lifetimes. How can these still be surviving, given that our earth has been around for billions of years?
The answer is that they are the daughters (or grand , or grand-grand or grand-grand-grand etc. daughters) of a very long
lived parent, which keeps producing a large family of offsprings. The best known family is the one of
whose half-life
of
is probably about half the estimated age of the earth. About half of the
that was present when the
earth was formed is still there, and the energy associated with its decay is most likely the source of the intense heat within the
earth's core.
Other sources of radioactive isotopes are the cosmic rays, i.e mostly high energy protons produced in various
cosmic events, that, when crossing the earth's path, collide with the air molecules. The continuous replenishment of one
specific radioactive isotope,
(
yr) is what has allowed the technique of carbon dating : under the
assumption that the ratio of
to
has remained constant in the environment over the last millennia, then living
organisms should contain in their tissues the two isotopes in a constant ratio. But,when they die (i.e. when they stop
exchanging carbon with the environment), their
content will gradually decay away. Measuring the leftover
to
ratio allows to determine for how long the organism has been dead...
Other isotopes rather than
can in principle be exploited for radioactive dating, with the obvious condition that the
isotope lifetime has to be of the same order as the time interval one wants to measure.
Units of (radio)activity:
For a given sample, the rate of decays taking place in a given time will depend on the size of the sample and on its lifetime.
Specifically, one could see (by means of calculus techniques) that the property of the exponential function discussed before
implies that, in any given time interval, the number of decays taking place is proportional to the number of unstable particle
initially present and to the time interval, i.e.
the minus sign representing the fact that the number of particles is decreasing. If we define the activity of a sample
to be the number of decays per unit time, one has immediately
activity =
The traditional unit for measuring the (radio)activity of a sample is the curie (Ci) = 3.7 x 10
disintegrations
per second. In the usual attempt to rationalize units, the SI unit for activity is the becquerel (Bq) = 1 disintegration per
second.
Radiation Detectors. Practically all radiation detectors rely on the interactions between the particles one wants to
detect and the atomic electrons of the detector material itself. This is why it is much more easy to detect charged rather than
neutral particles : to detect a neutron, one has to rely on the rare chance of the neutron "hitting" a proton within a
nucleus, so that what one detects is the recoil proton.
rays (i.e. photons), although neutral, can be detected more easily
than neutrons or other neutral particles, since
rays are in fact electromagnetic radiation, and they do interact (e.g.
by Compton scattering) with electrons, although the process is not as likely as the direct interaction betwen charged particles.
When a charged particle crosses a medium, it ionizes it (this is why one talks in general about ionizing radiation), leaving
a trail of free electrons + ions on its path. Several techniques (Geiger counters, proportional chambers, bubble and cloud
chambers, etc.) have been developed to detect these trails of electrons.
Alternatively, a charged particle might just excite the electrons of the detector material to higher orbits, and a small
light pulse will be generated when the electrons fall back to their original levels. A photomultiplier is then used to
convert the tiny light pulse into a detectable electric pulse.
Next: About this document ...
Sergio Conetti
2003-04-24